Next: Conversion of time averages
Up: The cavity system
Previous: The cavity system
Form of correlation spectrum and timescales
As presented in Section 2.1, the generalized time-dependent
`force' associated with the parameter
is
, where
is
a constant such that the time-average is zero.
It has the
-independent auto-correlation function
 |
|
|
(3.2) |
The subscript
, whenever used, suggests that the
average over initial conditions is of microcanonical
type, with energy
.
For the Hamiltonian (3.1) we can write
 |
|
|
(3.3) |
Recognizing
as the force on the gas particle,
we see that
is a train of spikes superimposed on a constant
average value (see Fig. 3.3a):
 |
|
|
(3.4) |
where
labels collisions:
is the time of a collision,
stands for
at the location
of a collision, and
is the normal component of the particle's collision velocity.
If the deformation is volume-preserving then
, otherwise it is
convenient to subtract the (constant) average value
.
The auto-correlation function
can be handled as a time-average of a single trajectory rather than
an ensemble-average over trajectories
(by ergodicity, as discussed in Section 2.1.6);
the resulting construction is
illustrated in Fig. 3.3b.
The forms of
and
its Fourier transform
are illustrated schematically
in Figs. 3.3c and 3.3d.
The removal of the average value of
ensures that there is
no
-function spike at
in
.
This is required so that
has a well-defined
limit, namely the fluctuations intensity
.
The auto-correlation function
consists of a
(`self')
peak due to the self-correlation of the
spikes, and of an additional smooth (`non-self') component due to
correlations between successive bounces.
(Note that in the hard walled limit the constant value
contributes nothing to the `self'
peak, so its only effect
is on the `non-self' component).
Thus two time scales
are involved: the short time scale is
in the hard wall limit,
and the other time scale is
, which
involves the time for succesive collisions with the deforming
part of the boundary.
Note that
can
be much larger than the
ballistic time
(the average time to cross
the billiard) in the case that only a small piece
of the boundary is being deformed.
The quantitative definition of the collision rate
is postponed to Section 3.2.
I shall be most interested in the noise intensity
defined by (2.14).
Observing that
is linear in
,
it follows that the noise intensity must (exactly) be a general quadratic functional
 |
(3.5) |
where the kernel
depends on both the
cavity shape and the particle energy
[120].
Furthermore, billiards
are scaling systems in the sense that a change in
leaves the
trajectories unchanged.
From this and (3.4) we have the scaling relation
,
where the scaled kernel depends entirely on the geometrical
shape of the cavity.
However, the reason for being interested in approximations for
is that the exact result for the kernel
is not analytic: it is very complicated to evaluate, and
involves a sum over all classical paths from
to
(see [120]).
Figure:
a) The fluctuating force
is a series of impulses
of maximum duration
.
In the hard-walled limit
.
The average value
has been subtracted.
b) The autocorrelation function
can be viewed
as the average projection of
the function
onto the
axis (the `self' components are shaded).
c) The autocorrelation function has a `self' peak of
half-width
, and becomes negligible beyond
the ergodic time
.
d) The noise power spectrum
. Its
limit
is equal to the area under
.
The power spectrum typically shows billiard-specific features
around the frequency
, where
is the ballistic time.
Note that in some examples
is much
longer than
.
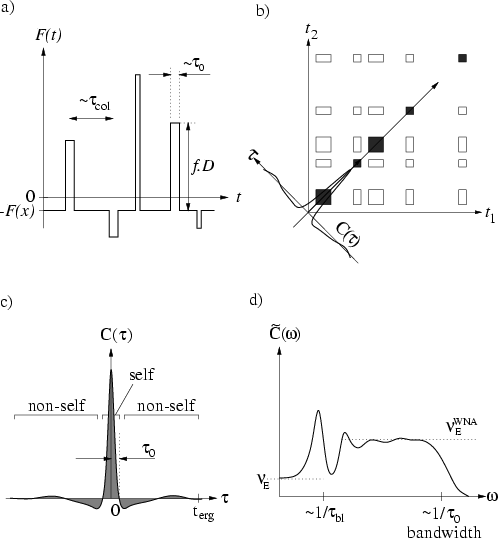 |
Next: Conversion of time averages
Up: The cavity system
Previous: The cavity system
Alex Barnett
2001-10-03